A Research team from New York University conducted an innovative study which merged contemporary imaging technology with large-scale molecular dynamics simulations to reveal the basic nucleation pathways behind crystal formation methods. The research discoveries show the fundamental structures of initial cluster formation together with established synthesis parameters that provide reliable reproduction conditions. As part of our ongoing coverage of breakthrough work in chemical science, we sat down with Shihao to explore his approaches, applications, and the future directions of his findings based on our earlier news report. The main research paper pertaining to this interview can be found below:
Zang, S., Paul, S., Leung, C. W., Chen, M. S., Hueckel, T., Hocky, G. M., & Sacanna, S. (2025). Direct observation and control of non-classical crystallization pathways in binary colloidal systems. Nature Communications, 16(1), 3645. https://doi.org/10.1038/s41467-025-58959-0
To better understand the system of their latest work, The research team has recommended the following two published papers from their group:
Polymer-attenuated Coulombic self-assembly (PACS) method to assemble oppositely charged colloidal particles into ionic crystals in aqueous solution:Hueckel, T., Hocky, G.M., Palacci, J. et al. Ionic solids from common colloids. Nature 580, 487–490 (2020). https://doi.org/10.1038/s41586-020-2205-0
‘Crystal Clear’ technique to track particles dynamically inside the ionic crystals: Zang, S., Hauser, A.W., Paul, S. et al. Enabling three-dimensional real-space analysis of ionic colloidal crystallization. Nat. Mater. 23, 1131–1137 (2024). https://doi.org/10.1038/s41563-024-01917-w
Other interesting publications from their lab can be found here: https://www.sacannalab.com/publications-year
Follow the main authors here:
The study’s other authors are Sanjib Paul, Cheuk Leung, Michael Chen, and Theodore Hueckel.
The following interview is presented unedited to preserve Shihao Zang’s original responses and offer an unfiltered glimpse into his innovative contributions to crystallisation science.
Acknowledgment from Shihao Zang and team: The research was supported by the US Army Research Office (W911NF-21-1-0011), the Simons Center for Computational Physical Chemistry at NYU (SCCPC, Simons Foundation 839534), and the National Institutes of Health (R35GM138312). Computational work was supported in part through the NYU IT High Performance Computing resources, services, and staff expertise, and simulations were partially executed on resources purchased by the SCCPC.
Could you describe the key steps in the nucleation and growth mechanism to form zangenite crystals, and how this pathway differs from classical colloidal crystallisation?
In the paper, we directly observed both classical and non-classical nucleation pathways, modulated by strength of particle interactions. Under weaker particle interactions, crystals nucleated directly from the gas phase via a classical mechanism. In contrast, stronger interactions promoted a non-classical, two-step pathway: initially, positively and negatively charged particles rapidly condensed into dense, liquid-like blobs. Crystallisation then initiated within these blobs and gradually transformed into fully ordered structures.
Since the interaction strength is highly sensitive to salt concentration, we developed a continuous dialysis setup for gradual and spatially controlled variation of the salt concentration. This was accomplished by diffusing salt from the sample into surrounding deionized water baths, with the dynamics modeled using Fick’s second law and COMSOL simulations.
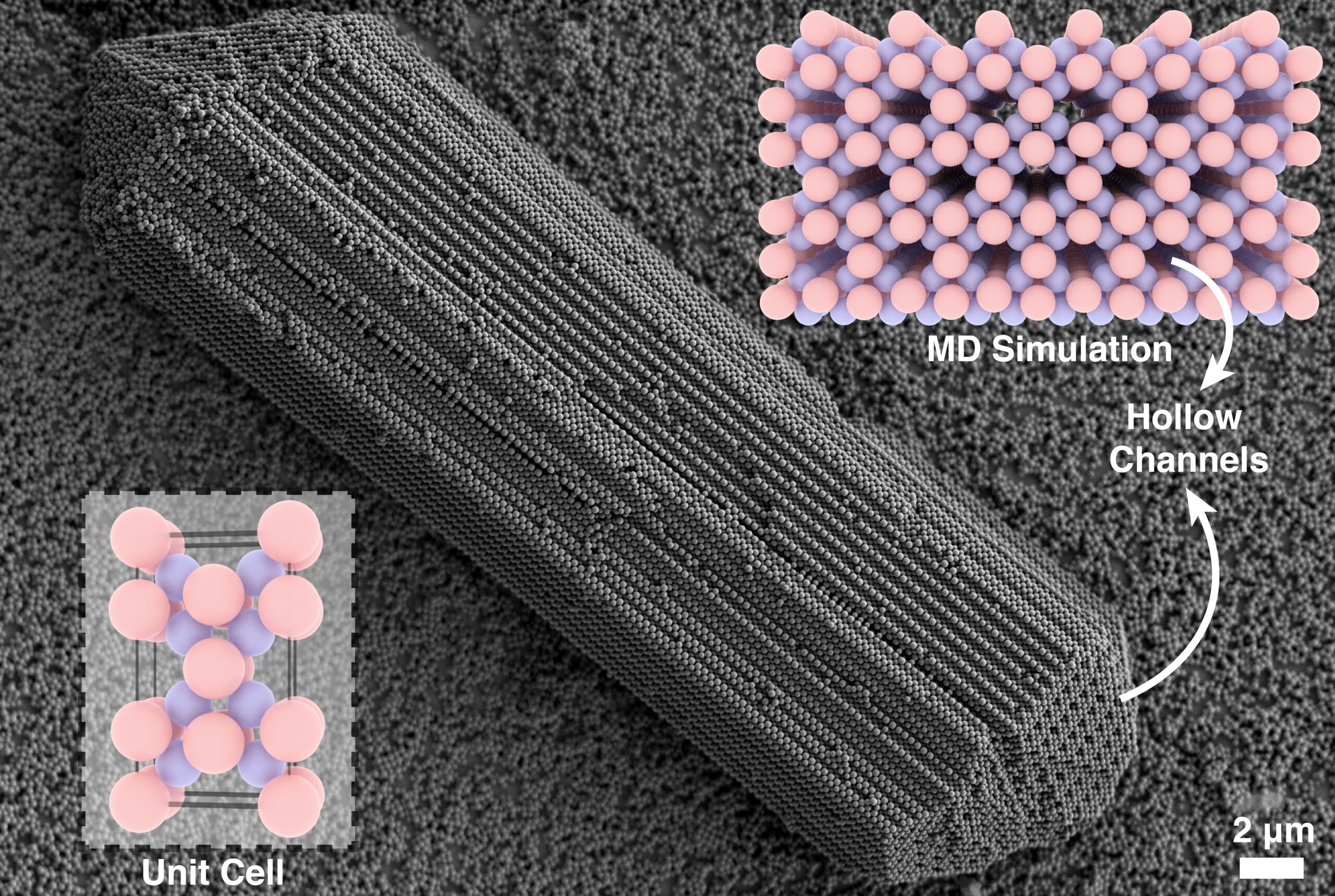
In this dialysis setup, Zangenite, a rod-shaped crystal, predominantly emerged near the sealed ends of the capillaries, often coexisting with a branched crystal yet to be identified in our lab. Based on our observations, Zangenite nucleates on negatively charged glass substrates through a classical pathway. A critical step in its formation is the establishment of the first two layers: the first layer consists of positively charged particles arranged in a coupled and separated spacing, while the second layer forms a characteristic zig-zag pattern of negatively charged particles. The emergence of this complex layered motif requires a slow evolution of interactions over extended time scales, which likely explains why Zangenite crystals are confined to regions where ionic strength changes most gradually. Following nucleation, Zangenite grows anisotropically into a rod-shaped morphology.
What microscopic imaging approaches did you rely on to track individual colloidal particles during crystallisation, and what challenges did you face in resolving zangenite’s hollow channels?
Crystallisation process with polystyrene (PS) particles was monitored under brightfield microscope for dynamic observation of the morphology change. However, resolving individual colloidal particles requires fixing and drying the assembled structures, followed by imaging with scanning electron microscopy (SEM).
For real-time single-particle tracking during crystallization, we typically rely on laser scanning confocal microscopy (LSCM) using low-refractive-index pentafluoropropyl methacrylate (PFPMA) particles, which are optically matched to the surrounding solvent. Unfortunately, we did not observe Zangenite under these in situ imaging conditions. This limitation arises because PFPMA is denser than PS, which enhances gravitational effects and likely inhibits the formation of open structures like Zangenite. As a result, SEM remains the only technique we have used to resolve Zangenite’s structure at the single-particle level.
When we first observed the rod-like morphology of Zangenite crystals under SEM, we initially suspected they might correspond to AlB₂-type structures previously reported in our lab, due to the similar shape. However, the presence of the unusual crystalline pattern of dark spots —hollow channels at the rod tips— prompted closer inspection. We modeled the packing of the AlB₂ unit cell using Blender and Mercury software, and found that the size ratio of the oppositely charged particles in this system made the formation of AlB₂ geometrically unachievable due to particle overlapping. This discrepancy led us to realize that this rod-shaped crystal was a previously unreported structure. Interestingly, it was the observation of those hollow channels that first alerted us to this misidentification, ultimately guiding us towards the discovery of Zangenite.
How did you integrate computational modelling or molecular dynamics simulations into your study, and what insights did they provide about the stability and formation conditions of zangenite?
As real-time 3D imaging using laser scanning confocal microscopy (LSCM) was not feasible for Zangenite, we turned to molecular dynamics (MD) simulations to gain insights into both the dynamic formation process and the structural details of the crystal. These simulations not only allowed us to observe the assembly pathway but also enabled precise reconstruction of the unit cell from the known (x, y, z) coordinates of individual particles.
In MD simulations, we used the experimentally matched particle sizes and number ratios of oppositely charged colloids, with a negatively charged wall at the bottom of the simulation box to mimic the experimental substrate. Under these conditions, the system reproducibly assembled rod-like crystals with hollow channels, consistent with the Zangenite morphology observed via SEM.
From the simulation data, we reconstructed the unit cell and identified the crystal as having a Cmmm (No. 65) space group, with an L3S4 stoichiometry (L for large, S for small particles). The unit cell dimensions were measured to be a = 254 nm, b = 509 nm, and c = 923 nm. The simulation trajectory also revealed the nucleation sequence: the first and second layers formed on the charged substrate via a classical mechanism, and the crystal subsequently grew anisotropically into its characteristic rod-like shape. We also captured the competition between CsCl-like and Zangenite structures. While both phases can emerge under similar conditions, the presence of a negatively charged wall significantly favors the nucleation and growth of Zangenite. Furthermore, seeded growth simulations without the charged wall demonstrated that Zangenite can continue to grow without structural collapse, suggesting that it is thermodynamically stable once nucleated.
Zangenite represents a previously unknown crystal type; what are its defining structural features at the colloidal scale, and how do those features translate into new material properties?
The first feature of Zangenite is the unit cell information with Cmmm symmetry, which underpins its directional growth and suggests potential for anisotropic mechanical or optical properties.
The second feature is the presence of hollow channels running along the crystal axis, resulting in a low packing density (~56%) and significant internal porosity. These characteristics make Zangenite a promising candidate for applications that benefit from lightweight, high-surface-area materials, such as filtration or selective transport.
Additionally, both experimental observations and molecular dynamics simulations show that Zangenite can serve as templates for heteroepitaxial growth, supporting the nucleation of secondary structures such as CsCl-like crystals and a flag-shaped crystalline extension. This ability to host multi-phase growth highlights Zangenite’s potential in hierarchical assembly and the design of composite or multifunctional materials.
How reproducible is the synthesis of pure zangenite, and which parameters (particle size distribution, solvent polarity, temperature) have the greatest impact on phase selectivity and crystal habit?
Within our current dialysis setup, we typically observe a mixture of five polymorphs—CsCl-like, Th₃P₄-like, Zangenite, flag-like, and branched crystals—coexisting under similar conditions. Achieving phase-pure Zangenite remains a challenge and likely requires more precise control over nucleation kinetics and a deeper understanding of the competing crystals.
From our observations, three parameters are particularly critical for reproducibly assembling Zangenite. First, a size asymmetry between the two particle types is essential, with the optimal size ratio near 0.8. Second, charge asymmetry plays a key role: the smaller particles must be positively charged, while the substrate should carry an opposite (negative) charge to stabilize the initial nucleating layers that define the Zangenite motif. Third, the absolute size of the building blocks significantly impacts the dimensionality of the assembled crystals. Smaller particles (~200 nm) favor the formation of well-defined, rod-shaped crystals, while larger particles (~500 nm) tend to assemble into only a few layers。
What potential applications are you most excited about for zangenite; be it in photonic materials, catalysis, or template-directed self-assembly, and have you begun any preliminary tests in these areas?
What excites us most about Zangenite is its hollow channel architecture and low packing density, which open up opportunities across several application areas. Fundamentally, gaining a deeper understanding of how such open-framework colloidal crystals form could inform the design of next-generation materials, including photonic bandgap materials, that are critical for technologies such as lasers, fiber-optic cables, and solar panels.
We have begun preliminary modeling to explore Zangenite’s photonic potential by calculating the band structure based on the resolved unit cell. Unfortunately, so far, no complete photonic bandgap has been observed. Nonetheless, the open geometry and anisotropic features remain promising, especially if functionalized or combined with high-index building blocks.
Beyond photonics, we see strong potential in template-directed self-assembly, where Zangenite could act as a scaffold for the growth of secondary materials or hierarchical composites. These directions are part of our ongoing and future investigations.
In this study, we focused primarily on oppositely charged particles with a size ratio of 0.81, which led to the discovery of Zangenite. Moving forward, we plan to expand our exploration to a wider range of size ratios using the same continuous dialysis setup. This may reveal additional, previously unobserved colloidal crystal structures. Once new assemblies are obtained, we will systematically analyze their unit cells and compare them to known atomic counterparts—or identify them as entirely novel structures.
On the theoretical side, we are developing a computational platform that mimics the experimental dialysis environment. This simulation tool is designed to provide predictive guidance on which size ratios and interaction conditions are most likely to yield specific crystal phases. By combining simulation and experiment in a feedback loop, we aim to build a predictive framework for crystal discovery.
While colloidal crystals have traditionally served as models for atomic solids, this methodology opens the possibility to go beyond mimicking and actually discover new structural motifs that remain unexplored even in atomic crystallography.
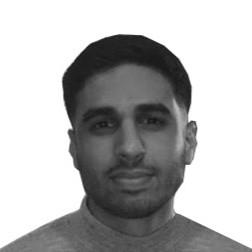
Hassan graduated with a Master’s degree in Chemical Engineering from the University of Chester (UK). He currently works as a design engineering consultant for one of the largest engineering firms in the world along with being an associate member of the Institute of Chemical Engineers (IChemE).